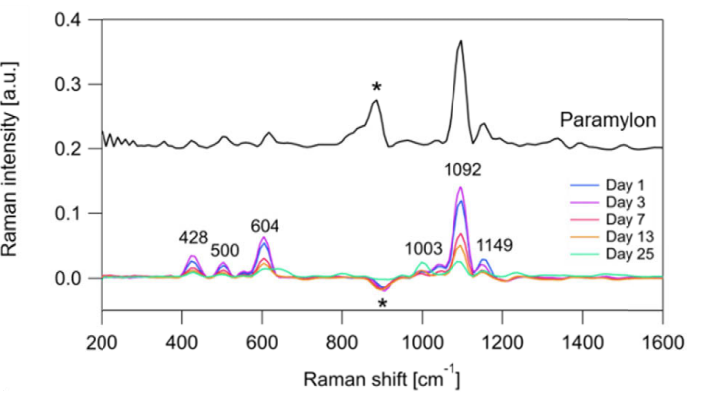
Detection of Intracellular Target Substances Using Raman Scattering Light
An important approach to identifying valuable E. gracilis involves detecting and visualizing whether the desired substances are present within cells and, if so, to what extent. While immunocytochemistry and staining reagents can label target substances within specific cells, methods requiring cell fixation are unsuitable for detecting intracellular target substances in live cells. Achieving this capability could facilitate real-time analysis of cells and the isolation of cells possessing target substances while alive, potentially leading to the acquisition of valuable cell strains.
By employing labeling for cell surface antigens or staining reagents designed for live cells, it is possible to detect target substances within living cells. However, challenges arise when specific antibodies are unavailable, toxicity of reagents cannot be ignored, or suitable staining reagents are non-existent. Addressing these issues, a method utilizing Raman scattering light without the need for special labeling has been developed to detect intracellular target substances.
Raman Shift of Paramylon
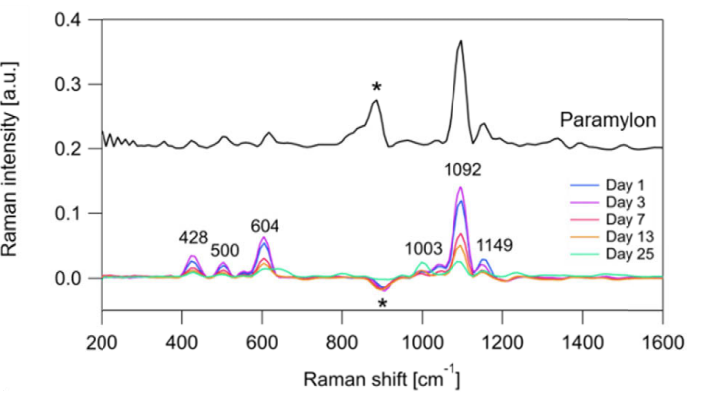
Figure 1 Raman Shift (Adapted from Reference 1)
When light is scattered upon interaction with matter, it produces light with slightly different wavelengths than the incident light (Raman scattering). Since Raman scattering light is unique to molecular structures, characteristic peaks are obtained corresponding to different substances (Raman shift). For instance, Figure 1 compares the Raman shift when light is irradiated on Paramylon (above) and E. gracilis (below) (Reference 1). Paramylon-specific peaks are observed in E. gracilis, indicating the presence of Paramylon within the cells. Furthermore, peak height (Raman intensity) indicates substance concentration, facilitating comparison of target substance quantities under different cultivation conditions (as shown in Figure 1).
In principle, any light can be used to obtain Raman scattering light, without requiring prior fixation or preparation. Imaging Raman scattering light signals allows visualization of target substances without labeling. However, performing live imaging of cells, especially mobile ones like E. gracilis, was challenging. This was due to the weak scattered light and the time required for detection at various wavelengths, resulting in signal imaging taking several hours (Reference 2).
Advancements in technology led to successful observation of living E. gracilis cells in 2016. Induced Raman scattering (SRS) microscopy, which detects variations in light induced between two different light sources when they hit the target substance, was used (Reference 3). The video below demonstrates label-free visualization of lipids (blue), Paramylon (red), and chloroplasts (green) in moving E. gracilis cells (Adapted from Reference 3).
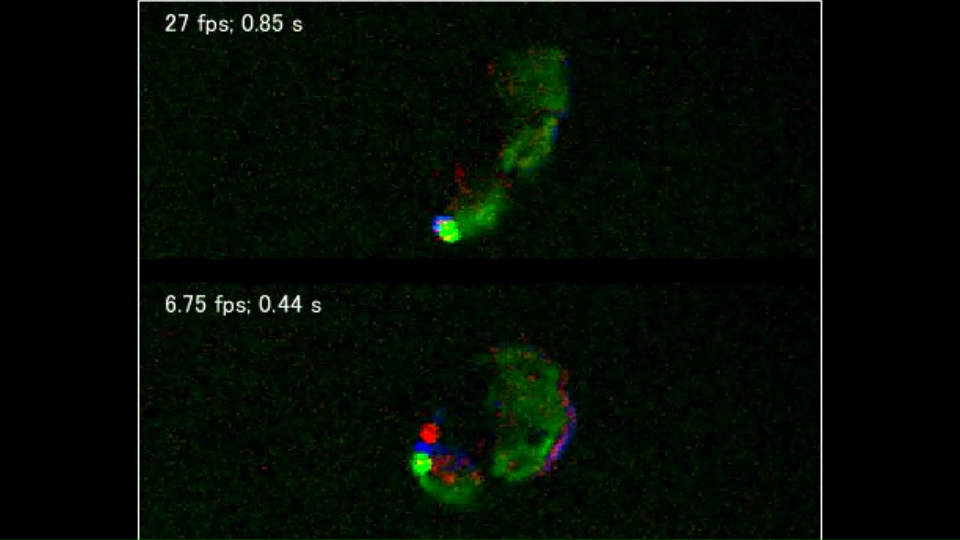
In 2020, Coherent Anti-Stokes Raman Scattering (CARS), an alternative signal enhancement method to SRS, was used in combination with a flow cytometer to enable scalable analysis of E. gracilis (Figure 2, Reference 1). With a speed of 20-80 cells/second, it can analyze up to 10,000 cells in just 2 minutes. Developing this technology for cell sorting may eventually allow isolation of target cells without labeling.
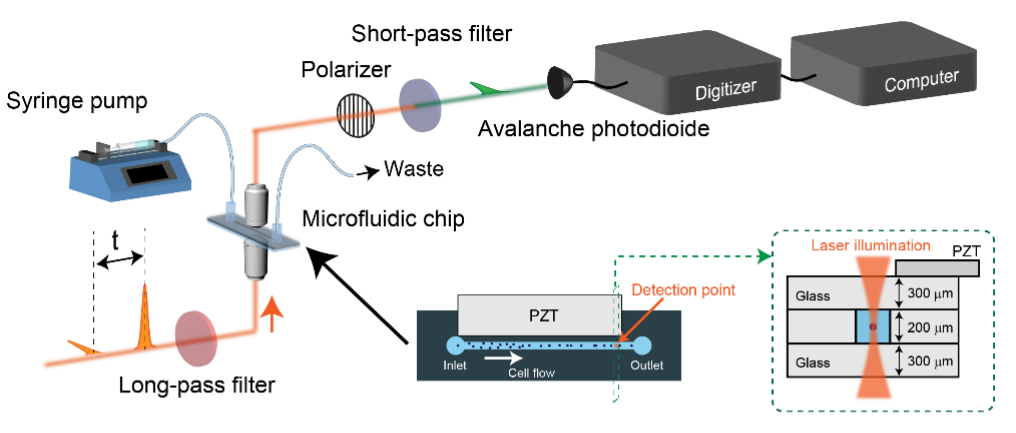
Figure 2 FT-CARS Flow Cytometer (Adapted from Reference 1)
Cells isolated and cultured are generally considered as individual cell populations. However, reports have indicated the presence of subpopulations due to differences in gene expression and phenotype (References 4, 5). Advancements in detecting target substances through Raman scattering light and speeding up detection could potentially reveal various E. gracilis cell states within a uniform population.
References:
- Hiramatsu, K. et al. Large-scale label-free single-cell analysis of paramylon in Euglena gracilis by high-throughput broadband Raman flow cytometry. Biomed. Opt. Express 11, 1752 (2020).
- Ozeki, Y. Induced Raman scattering for biological imaging. Biophysical Reviews 6, 311-314 (2014).
- Wakisaka, Y. et al. Probing the metabolic heterogeneity of live Euglena gracilis with stimulated Raman scattering microscopy. Nat. Microbiol. 1, 1–4 (2016).
- Lidstrom, M. E. & Konopka, M. C. The role of physiological heterogeneity in microbial population behavior. Nat. Chem. Biol. 6, 705–712 (2010).
- Chen, C. et al. Proteomic study uncovers molecular principles of single-cell-level phenotypic heterogeneity in lipid storage of Nannochloropsis oceanica. Biotechnol. Biofuels 12, 1–14 (2019).